Chapter XI THE ELECTROMAGNETIC PULSE AND ITS EFFECTS
ORIGIN AND NATURE OF THE EMP
INTRODUCTION
11.01 Explosions of conventional high explosives can produce electromagnetic signals and so the generation of an electromagnetic pulse (EMP) from a nuclear detonation was expected. However, the extent and potentially serious nature of EMP effects were not realized for several years. Attention slowly began to focus on EMP as a probable cause of malfunction of electronic equipment during atmospheric nuclear tests in the early 1950's. Induced currents and voltages caused unexpected equipment failures and subsequent analysis disclosed the role of EMP in such failures. Finally, around 1960 the possible vulnerability of various civilian and military electrical and electronic systems to EMP was recognized. At about the same time it became apparent that the EMP could be used in the long-range detection of nuclear detonations.
11.02 For the foregoing reasons, theoretical and experimental efforts have been made to study the EMP and its effects. A limited amount of data had been gathered when aboveground testing was halted in 1962. Subsequently, reliance has been placed on underground testing, analysis of existing atmospheric test data, non nuclear simulation, and theoretical calculations. Extended efforts have been made to improve theoretical models and to develop associated computer codes for predictive studies. In addition, simulators have been developed which are capable of producing representative pulses for system coupling and response studies.
11.03 Nuclear explosions of all types - from underground to high altitudes - are accompanied by an EMP, although the intensity and duration of the pulse and the area over which it is effective vary considerably with the location of the burst point. The strongest electric fields are produced near the burst by explosions at or near the earth's surface, but for those at high altitudes the fields at the earth's surface are strong enough to be of concern for electrical and electronic equipment over a very much larger area.
11.04 The nuclear EMP is a time varying electromagnetic radiation which increases very rapidly to a peak and then decays somewhat more slowly. The radiation has a very broad spectrum of frequencies, ranging from very low to several hundred megahertz but mainly in the radiofrequency (long wavelength) region (Fig. 1.74). Furthermore, the wave amplitude (or strength) of the radiation varies widely over this frequency range. Because the EMP is a very complex phenomenon dependent upon the conditions of the burst, the descriptions given in this chapter are largely qualitative and sometimes over simplified. They should, however, provide a general indication of the origin and possible effects of the EMP.
DEVELOPMENT OF AN ELECTRIC FIELD
11.05 The instantaneous (or prompt) gamma rays emitted in the nuclear reactions and those produced by neutron interactions with weapon residues or the surrounding medium (Fig. 8.14) are basically responsible for the processes that give rise to EMP from bursts in the lower atmosphere. The gamma rays interact with air molecules and atoms, mainly by the Compton effect (§ 8.89), and produce an ionized region surrounding the burst point (§ 8.17). In EMP studies this is called the “deposition region.” The negatively charged electrons move outward faster than the much heavier positively charged ions and as a result there is initially a separation of charges. The region nearer to the burst point has a net positive charge whereas that farther away has a net negative charge. This separation of charges produces an electric field which can attain its maximum value in about 10-8 second, i.e., one hundredth part of a microsecond(§ 1.54 footnote).
11.06 If the explosion occurred in a perfectly homogeneous (constant density) atmosphere and the gamma rays were emitted uniformly in all directions, the electric field would be radial and spherically symmetric, i.e., it would have the same strength in all directions outward from the center (Fig. 11.06a). There would then be no electromagnetic energy radiated from the ionized deposition region. In practice, however, such an ideal situation does not exist; there is inevitably some condition, such as differences in air density at different levels, proximity of the earth's surface, the non uniform configuration of the exploding weapon (including auxiliary equipment, the case, or the carrying vehicle), or even variations in the water vapor content of the air, that will interfere with the symmetry of the ionized. region. If the burst occurs at or near the earth's surface, the departure from spherical symmetry will clearly be considerable. In all these circumstances, there is a net vertical electron current generated within the ionized deposition region (Fig. 11.06b). The time-varying current results in the emission of a short pulse of electromagnetic radiation which is strongest in directions perpendicular to the current; this is the EMP. In a high-altitude explosion, the EMP arises in a somewhat different manner, as will be seen shortly.
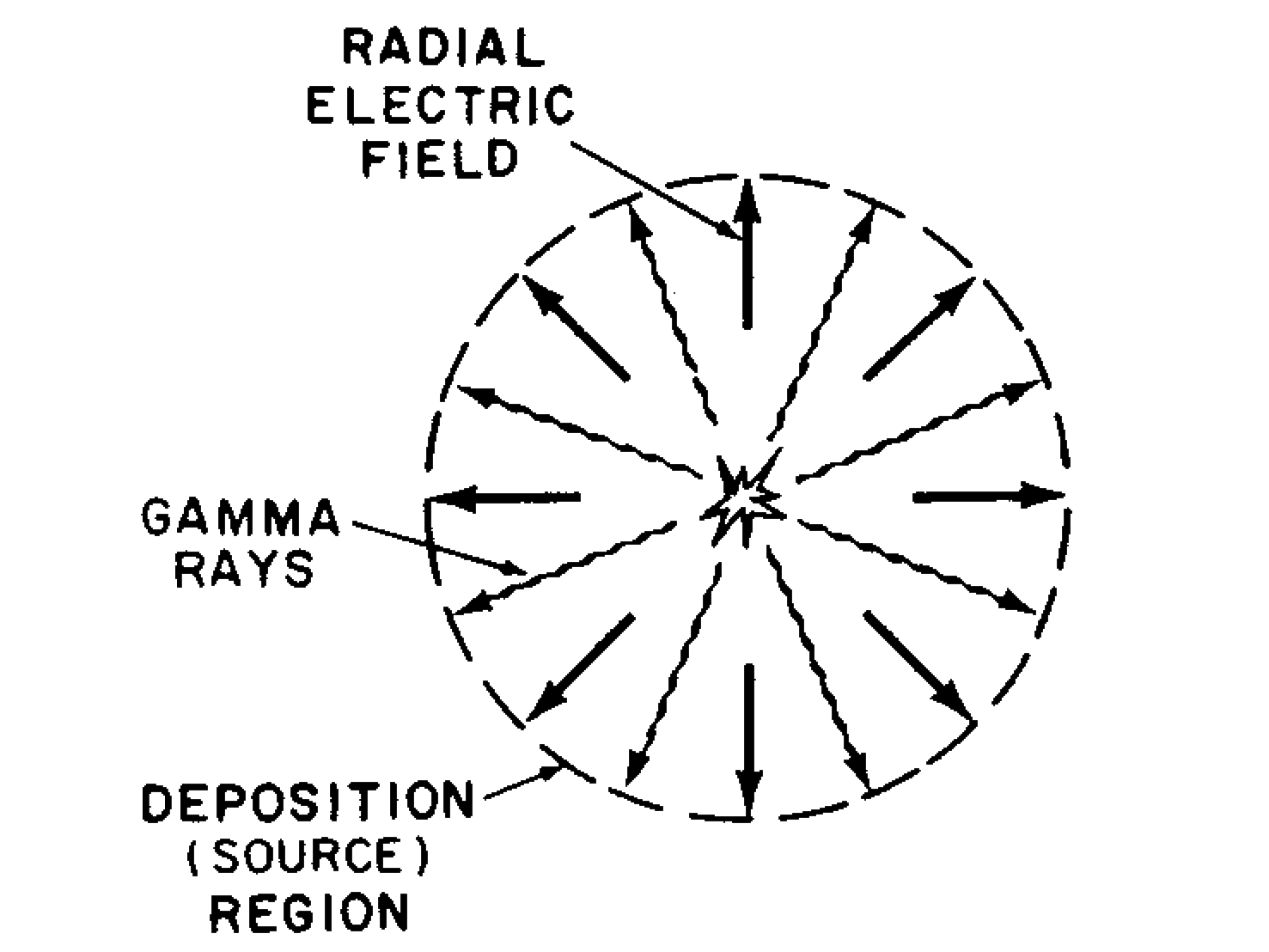
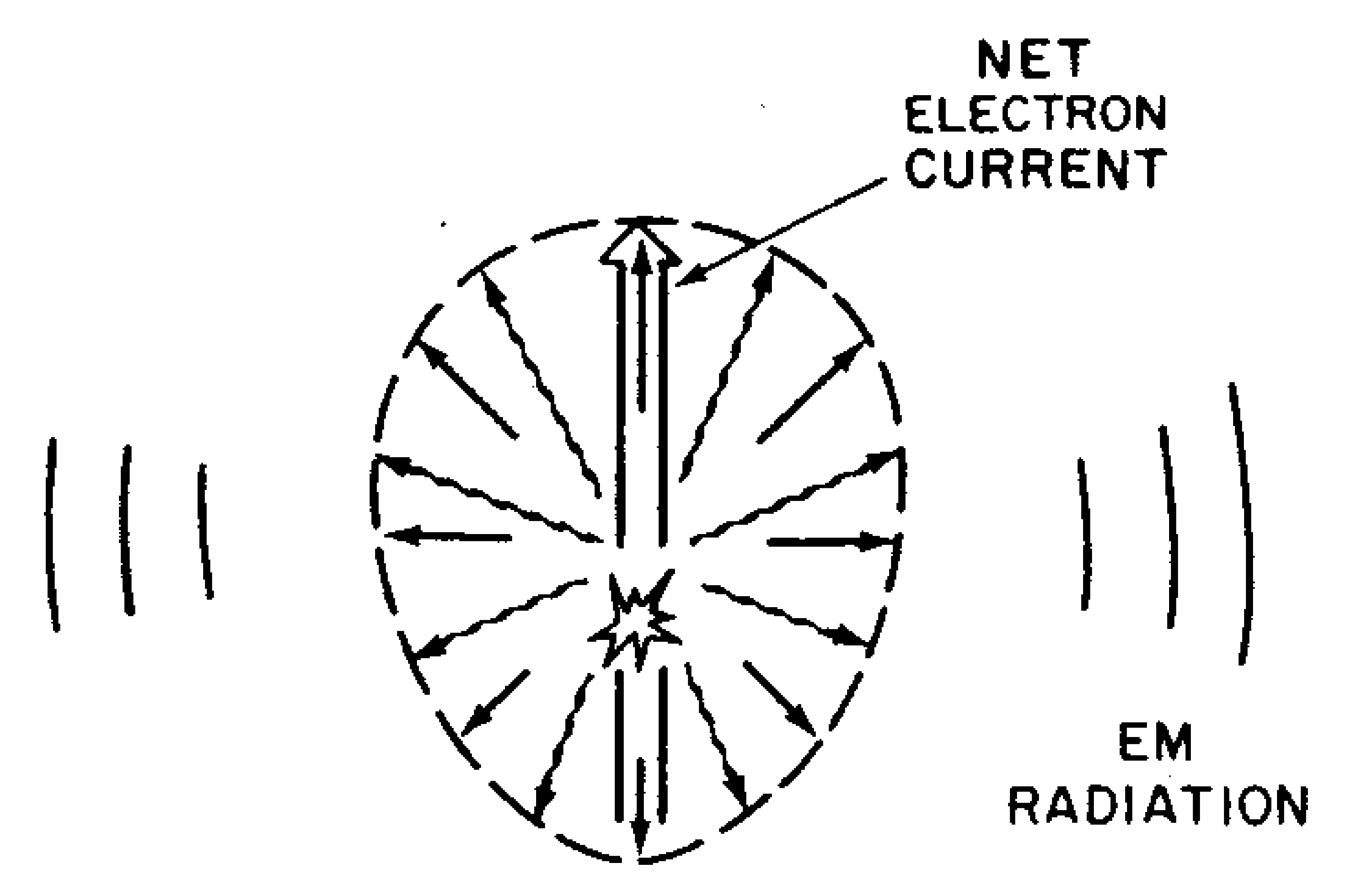
NATURE OF THE EMP
11.07 After reaching its maximum in an extremely short time, the electric field strength falls off and becomes quite small in a few tens of microseconds. In spite of the short duration of the pulse, it carries a considerable amount of energy, especially if the exploding weapon has a yield in the megaton range. As it travels away from the burst point at the speed of light, as do all electromagnetic waves (§ 1.73), the radiation can be collected by metallic and other conductors at a distance, just as radio waves are picked up by antennas. The energy of the radiation can then be converted into strong electric currents and high voltages. Electrical and electronic equipment connected to (or associated with) the collectors may thus suffer severe damage. The consequences could be serious for any system that relies on such equipment, e.g., commercial electric power generation and distribution systems, telecommunications, i.e., radio, radar, television, telephone, and telegraph systems, and electronic computers.
11.08 In a crude sense, the EMP radiations are somewhat similar to the familiar radio waves, although there are some important differences. Radio transmitters are designed to produce electromagnetic waves of a particular frequency (or wavelength), but the waves in the EMP have a wide range of frequencies and amplitudes. Furthermore, the strength of the electric fields associated with the EMP can be millions of times greater than in ordinary radio waves. Nevertheless, in each case, the energy of electromagnetic waves is collected by a suitable antenna (or conductor) and transferred to attached or adjacent equipment. The energy from the EMP is received in such a very short time, however, that it produces a strong electric current which could damage the equipment. An equal amount of energy spread over a long period of time, as in conventional radio reception, would have no harmful effect.
11.09 The characteristics of the EMP depend to a great extent on the weapon yield and height of burst. For explosions in the atmosphere at altitudes of a few miles, the deposition region will have a radius of about 3 miles, but it will increase to roughly 9 miles with increasing height of the burst point up to altitudes of approximately 19 miles. In this altitude range, the difference in air density across the vertical dimension of the deposition region will not be large and so the EMP effect will be moderate. In addition to the EMP arising from air density asymmetries, a short pulse is emitted in a manner similar to that described in § 11.14 for high-altitude bursts. The electric fields produced on the ground from air bursts between a few miles and about 19 miles altitude will be less than those radiated from surface (or near-surface) and high-altitude explosions. These latter two types of nuclear explosions will be considered briefly here, and more will be said later about them and about air bursts (§ 11.66 et seq.).
EMP IN SURFACE BURSTS
11.10 The mechanism of EMP formation is different in explosions at (or near) the surface and at high altitudes. In a surface burst, those gamma rays that travel in a generally downward direction are readily absorbed in the upper layers of the ground and there is essentially no charge separation or electric field in this direction. The gamma rays moving outward and upward, however, produce ionization and charge separation in the air. Consequently, there is a net vertical electron current (Fig. 11.10). As a result, the ionized deposit ion (source) region is stimulated to emit much of its energy as an electromagnetic pulse in the radiofrequency spectrum.
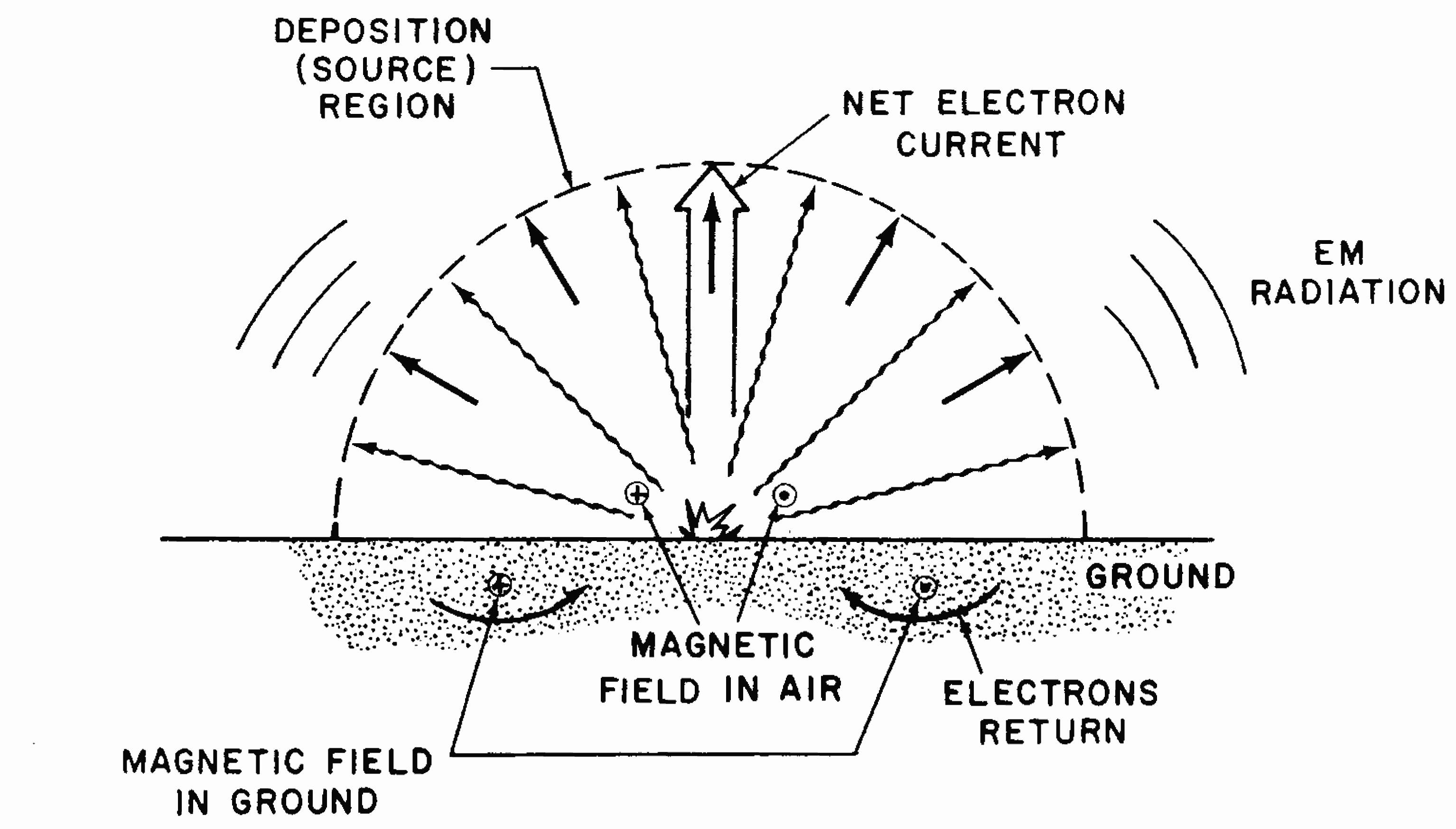
11.11 Since the ground is a relatively good conductor of electricity, it provides an alternative path for the electrons to return from the outer part of the deposition region toward the burst point where the positively charged ions, which have been left behind, predominate. Electric currents thus flow in the ground and generate strong magnetic fields in the region of the surface burst point.
11.12 The electric field produced in a surface burst is very strong but the radiated field falls off with increasing distance from the deposition region, at first quite rapidly and then somewhat less so. The potential hazard to electrical and electronic equipment from the EMP will thus be greatest within and near the deposition region which may extend over a radius around ground zero of about 2 to 5 miles, depending on the explosion yield. In this area, structures in which equipment is housed may suffer severe damage, especially from high-yield explosions, unless they are blast resistant. However, the threat to electrical and electronic systems from a surface-burst EMP may extend as far as the distance at which the peak overpressure from a 1-megaton burst is 2 pounds per square inch, i.e., 8 miles (see Chapter III). The degree of damage, if any, will depend on the susceptibility of the equipment and the extent of shielding (§ 11.33 et seq.).
EMP IN HIGH-ALTITUDE BURSTS
11.13 If the nuclear burst is at an altitude above about 19 miles, the gamma rays moving in an upward direction will enter an atmosphere where the air density is so low that the rays travel great distances before being absorbed. On the other hand, the gamma rays emitted from the explosion in a generally downward direction will encounter a region where the atmospheric density is increasing. These gamma rays will interact with the air molecules and atoms to form the deposition (or source) region for the EMP (Fig. I1.13). This roughly circular region may be up to 50 miles thick in the center, tapering toward the edge, with a mean altitude of about 25 to 30 miles. It extends horizontally for great distances which increase with the energy yield and the height of the burst point (see Figs. 11.70a and b).
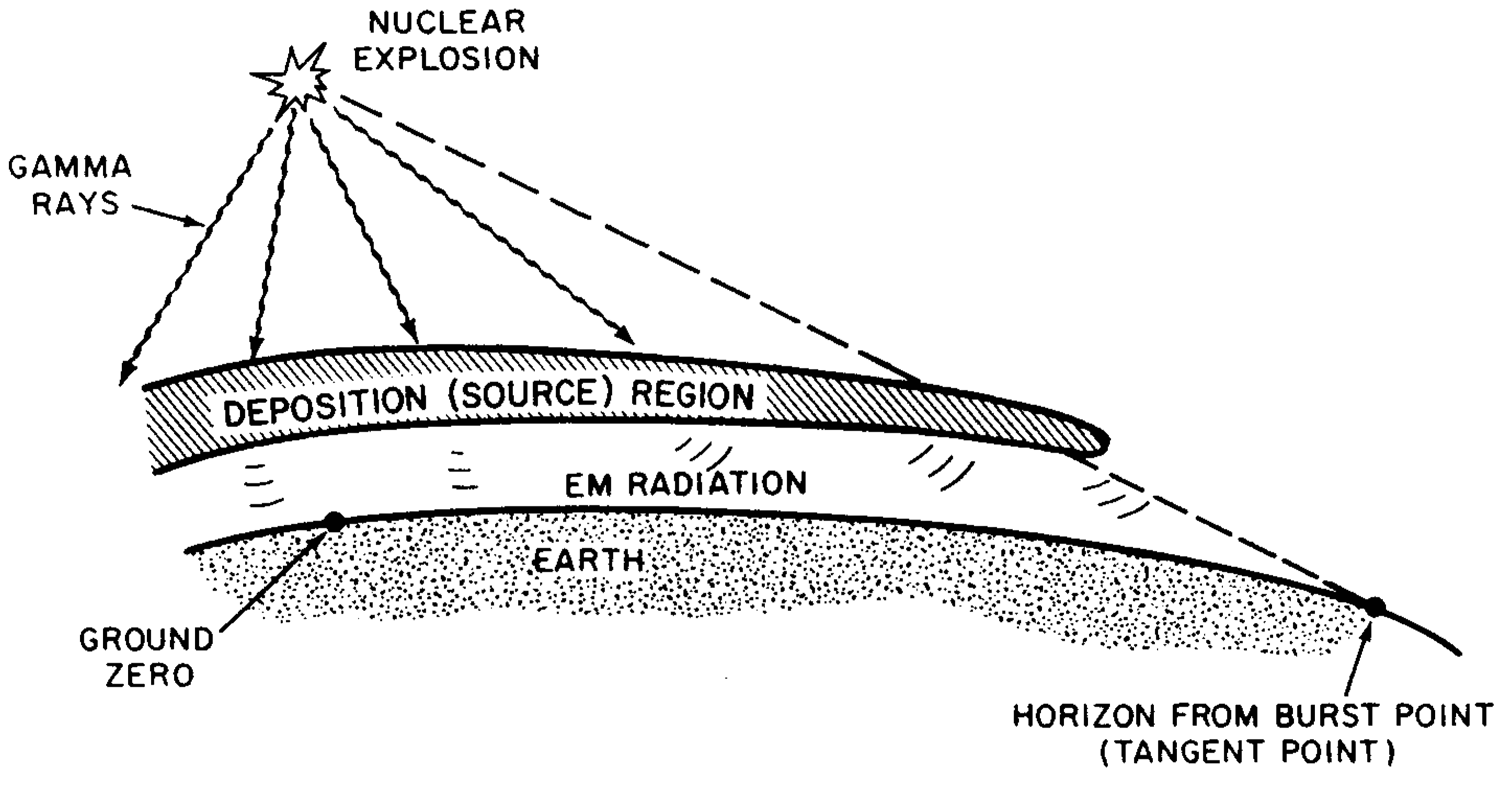
11.14 In the deposition region the gamma rays produce Compton electrons by interactions in the air; these electrons are deflected by the earth's magnetic field and are forced to undergo a turning motion about the field lines. This motion causes the electrons to be subjected to a radial acceleration which results, by a complex mechanism, in the generation of an EMP that moves down toward the earth. The pulse rises to a peak and then decreases, both taking place more rapidly than for a surface burst; as a result more of the electromagnetic energy appears in the higher frequency range (§ 11.63). The strength of the electric field observed at the surface from a high-altitude explosion is from one-tenth to a hundredth of the field within the source region from a surface burst. However, in a surface burst the radiated field strength drops off rapidly with distance outside this region and is then smaller than for a high-altitude burst. In the latter case, the radiated field does not vary greatly over a large area on the ground or in the atmosphere above the ground. The electric field is influenced by the earth's magnetic field, but over most of the area affected by the EMP, the electric field strength varies by not more than a factor of two for explosions with yields of a few hundred kilotons or more (§ 11.73).
11.15 For an explosion of high yield at a sufficient altitude, the area covered by the high-frequency EMP extends in all directions on the ground as far as the line-of-sight, i.e., to the horizon, from the burst point (see Fig. 11.13). The lower frequencies will constitute a significant pulse extending even beyond the horizon. For a nuclear explosion at an altitude of 50 miles, for example, the affected area on the ground would have a radius of roughly 600 miles and for an altitude of l00 miles the ground radius would be about 900 miles. For an explosion at 200 miles above the center of the (conterminous) United States, almost the whole country, as well as parts of Canada and Mexico, could be affected by the EMP. Thus, for a high-altitude burst, the damage could conceivably extend to distances from ground zero at which all other effects, except possibly eye injury at night (§ 12.79 et seq.), would be negligible. Furthermore, because the radiations travel with the speed of light, the whole area could be affected almost simultaneously by the EMP from a single high-altitude nuclear explosion.
COLLECTION OF EMP ENERGY
11.16 For locations that are not within or close to the deposition region for a surface or air burst, both the amount and rate of EMP energy received per unit area on or near the ground will be small, regardless of the type of nuclear explosion. Hence, for damage to occur to electrical or electronic systems, it would usually be necessary for the energy to be collected over a considerable area by means of a suitable conductor. In certain systems, however, sufficient energy, mainly from the high-frequency components of the EMP, may be collected by small metallic conductors to damage very sensitive components(§ 11.31). The energy is then delivered from the collector (antenna) in the form of a strong current and voltage surge to attached equipment. Actually, the equipment does not have to be attached directly to the collector; the EMP energy can be coupled in other ways(§ 11.27). For example, it is possible for an electric current to be induced or for a spark to jump from the conductor which collects the EMP energy to an adjacent conductor, not connected to the collector, and thence to a piece of equipment.
11.17 The manner in which the electromagnetic energy is collected from the EMP is usually complex, because much depends on the size and shape of the collector, on its orientation with respect to the source of the pulse, and on the frequency spectrum of the pulse. As a rough general rule, the amount of energy collected increases with the dimensions of the conductor which serves as the collector (or antenna). Typical effective collectors of EMP energy are given in Table 11.17. Deeply buried cables, pipes, etc., are generally less effective than overhead runs because the ground provides some shielding by absorbing the high frequency part of the energy (see, however, § 11.68).
|
SUMMARY OF EMP DAMAGE AND PROTECTION
11.18 The sensitivity of various systems and components to the EMP has been studied by means of simulators which generate sharp pulses of electromagnetic radiation (§ 11.41 et seq.). The results are not definitive because the amount of EMP energy delivered to a particular component would depend on the details of the circuit in which it is connected. Nevertheless, certain general conclusions seem to be justifiable. Computers and other equipment having solid-state components are particularly sensitive. Since computers are used extensively in industry and commerce, including electrical distribution and communications systems, the consequence of operational failure could be very serious. Vacuum-tube equipment (with no solid-state components) and low current relays, switches, and meters, such as are used in alarm and indicator systems, are less susceptible. The least susceptible electrical components are motors, transformers, circuit breakers, etc., designed for high-voltage applications. The threat to any component, regardless of its susceptibility to operational upset (temporary impairment) or damage, is increased if it is connected (or coupled) to a large collector. Conversely, the danger is diminished if the collector is small. Thus, although transistorized circuits are generally sensitive to the EMP, portable (battery operated) radios with very short “whip” or ferrite core antennas are not readily damaged unless they are close to a collector. Disconnection of a piece of equipment from the electric power main supply will decrease the energy collected, but this is not always feasible because it would deny use of the equipment.
11.19 Various means are possible for protecting or “hardening” equipment against damage by the EMP. Such protection is generally difficult for existing systems, but it can be built into new systems. Some of the approaches to hardening which have been proposed are the following: metal shields to prevent access of the radiation, good grounding to divert the large currents, surge arrestors similar to those used for lightning protection, and proper wiring arrangements. Finally, components that are known to be susceptible to damage by sharp pulses of electromagnetic energy should be eliminated. A further discussion of these procedures is given later in this chapter (§ 11.33 et seq.).
11.20 Except for locations close to a surface burst, where other effects would dominate in any event, the EMP radiation from a nuclear explosion is expected to be no more harmful to people than a flash of lightning at a distance. Tests on monkeys and dogs have shown that there are no deleterious effects from pulses administered either singly or repetitively over a period of several months. However, a person in contact with an effective collector of EMP energy, such as a long wire, pipe, conduit, or other sizable metallic object listed in Table 11.17, might receive a severe shock.
SYSTEM-GENERATED EMP11.21 In addition to the EMP arising from the interaction of gamma rays from a nuclear explosion with the atmosphere (or the ground), another type of electromagnetic pulse, called the “system-generated EMP” (or SGEMP), is possible. This term refers to the electric field that can be generated by the interaction of nuclear (or ionizing) radiations, particularly gamma rays and X rays, with various solid materials present in electronic systems. The effects include both forward- and backscatter emission of electrons and external and internal current generation.
11.22 The system-generated EMP is most important for electronic components in satellites and ballistic systems, above the deposition region, which would be exposed directly to the nuclear radiations from a high-altitude burst. The system-generated EMP can also be significant for surface and moderate-altitude bursts if the system is within the deposition region but is not subject to damage by other weapons effects. This could possibly occur for surface systems exposed to a burst of relatively low yield or for airborne (aircraft) systems and bursts of higher yield.
11.23 The system-generated EMP phenomenon is actually very complex, but in simple terms it may be considered to be produced in the following manner. The solid material in an electronic system or even in the shielding designed to protect the system from the external EMP contains atoms which are heavier than those present in the air. Consequently, interaction with gamma rays and high-energy X rays will produce electrons by both the Compton and photoelectric effects (§ 8.89 et seq.). These electrons can, in turn, interact with the solid material to release more electrons, called secondary electrons, by ionization. Such electrons as are produced, directly or indirectly, close to and on both faces of the solid material and have a velocity component perpendicular to the surface, will be emitted from the surface of the material. As a result, an electric field is generated near the surface. There are other effects, but they need not be considered here.
11.24 If the component has a cavity (or space) in which the gas pressure is very low, less than about 10-3 millimeters of mercury, very high electric fields - about 100,000 to a million volts per meter - can occur near the interior walls. At higher gas pressures, however, the electrons cause substantial ionization of the gas, e.g., air, thereby releasing low-energy (secondary) electrons. The relatively large number of secondary (conduction) electrons form a current which tends to cancel the electric field, thus enabling the high-energy electrons to move across the cavity more easily.
11.25 The electric fields generated near the walls by direct interactions of ionizing radiations with the materials in an electronic system can induce electric currents in components, cables, ground wires, etc. Large currents and voltages, capable of causing damage or disruption, can be developed just as with the external EMP. Because of the complexity of the interactions that lead to the system-generated EMP, the effects are difficult to predict and they are usually determined by exposure to radiation pulses from a device designed to simulate the EMP radiation from a nuclear explosion (§ 11.42).
EMP EXPERIENCE IN HIGH-ALTITUDE TESTS
11.26 The reality of damage to electrical and electronic equipment by the EMP has been established in various nuclear tests and by the use of EMP simulators. A number of failures in civilian electrical systems were reported to have been caused by the EMP from the high-altitude test explosions conducted in the Johnston Island area of the Pacific Ocean in 1962. One of the best authenticated cases was the simultaneous failure of 30 strings (series-connected loops) of street lights at various locations on the Hawaiian island of Oahu, at a distance of some 800 miles from ground zero. The failures occurred in devices called “fuses” which are installed across the secondaries of transformers serving these strings; the purpose of the fuses is to prevent damage to the lighting system by sudden current surges. Similar fuses associated with individual street lights were not affected. It was also reported that “hundreds” of burglar alarms in Honolulu began ringing and that many circuit breakers in power lines were opened. These occurrences probably resulted from the coupling of EMP energy to the lines to which the equipment was connected and not to failure of the devices themselves. No serious damage occurred since these items are among the least susceptible to the EMP (§ 11.18).
EMP DAMAGE AND PROTECTION1
COUPLING OF EMP ENERGY
11.27There are three basic modes of coupling of the EMP energy with a conducting system; they are electric induction, magnetic induction, and resistive coupling (sometimes referred to as direct charge deposition). In electric induction a current is induced in a conductor by the component of the electric field in the direction of the conductor length. Magnetic induction occurs in conductors that form a closed loop; the component of the magnetic field perpendicular to the plane of the loop causes current to flow in the loop. The form of the loop is immaterial and any connected conductors, even the reinforcing bars in concrete, can constitute a loop in this respect. Resistive coupling can occur when a conductor is immersed in a conducting medium, such as ionized air, salt water, or the ground. If a current is induced in the medium by one of the coupling modes already described, the conductor forms an alternative conducting path and shares the current with the medium.
11.28 If the EMP wave impinges upon the ground, a part of the energy pulse is transmitted through the air ground surface whereas the remainder is reflected. An aboveground collector, such as an overhead power line or a radio antenna tower, can then receive energy from both the direct and reflected pulses. The net effect will depend on the degree of overlap between the two pulses. The EMP transmitted into the ground can cause a current to flow in an underground conductor either by induction or by resistive coupling.
11.29 The coupling of electromagnetic energy to a conductor is particularly efficient when the maximum dimension is about the same size as the wavelength of the radiation. The conductor is then said to be resonant, or to behave as an antenna, for the frequency corresponding to this wavelength. Since EMP has a broad spectrum of frequencies, only a portion of this spectrum will couple most efficiently into a specific conductor configuration. Thus, a particular collection system of interest must be examined with regard to its overall configuration as well as to the component configuration. Most practical collector systems, such as those listed in Table 11.17, are complex and the determination of the amount of EMP energy collected presents a very difficult problem. Both computer methods and experimental simulation are being used to help provide a solution.
COMPONENT AND SYSTEM DAMAGE
11.30 Degradation of electrical and electronic system performance as a result of exposure to the EMP may consist of functional damage or operational upset. Functional damage is a catastrophic failure that is permanent; examples are burnout of a device or component, such as a fuse or a transistor, and inability of a component or subsystem to execute its entire range of functions. Operational upset is a temporary impairment which may deny use of a piece of equipment from a fraction of a second to several hours. Change of state in switches and in flip-flop circuits are examples of operational upset. The amount of EMP energy required to cause operational upset is generally a few orders of magnitude smaller than for functional damage.
11.31 Some electronic components are very sensitive to functional damage (burnout) by the EMP. The actual sensitivity will often depend on the characteristics of the circuit containing the component and also on the nature of the semiconductor materials and fabrication details of a solid-state device. In general, however, the components listed in Table 11.31 are given in order of decreasing sensitivity to damage by a sharp pulse of electromagnetic energy. Tests with EMP simulators have shown that a very short pulse of about 10-7 joule may be sufficient to damage a microwave semiconductor diode, roughly 5 × 10-2 joule will damage an audio transistor, but 1 joule would be required for vacuum tube damage. Systems using vacuum tubes only would thus be much less sensitive to the EMP than those employing solid-state components. The minimum energy required to damage a microammeter or a low current relay is about the same as for audio transistors.
|
11.32 As seen earlier, the EMP threat to a particular system, subsystem, or component is largely determined by the nature of the collector (antenna). A sensitive system associated with a poor collector may suffer less damage than a system of lower sensitivity attached to a more efficient collector. Provided the EMP energy collectors are similar in all cases, electrical and electronic systems may be classified in the manner shown in Table 11.32. However, the amount of energy collected is not always a sufficient criterion for damage. For example, an EMP surge can sometimes serve as a trigger mechanism by producing arcing or a change of state which, in tum, allows the normal operating voltage to cause damage to a piece of equipment. Thus, analysis of sensitivity to EMP may require consideration of operational upset and damage mechanisms in addition to the energy collected.
Most Susceptible
Less Susceptible
Least Susceptible
|
PROTECTIVE MEASURES
11.33 A general approach to the examination of a system with regard to its EMP vulnerability might include the following steps. First, information concerning the system components and devices is collected. The information is categorized into physical zones based on susceptibility and worst-case exposure for these items. It must be borne in mind in this connection that energy collected in one part of a system may be coupled directly or indirectly (by induction) to other parts. By using objective criteria, problem areas are identified, analyzed, and tested. Suitable changes are made as necessary to correct deficiencies, and the modified system is examined and tested. The approach may be followed on proposed systems or on those already existing, but experience indicates that the cost of retrofitting EMP protection may often be prohibitive. Consequently, it is desirable to consider the vulnerability of the system early during the design stage.
11.34 A few of the practices that may be employed to harden a system against EMP damage are described below. The discussion is intended to provide a general indication of the techniques rather than a comprehensive treatment of what is a highly technical and specialized area. Some of the methods of hardening against the EMP threat are shielding, proper circuit layout, satisfactory grounding, and various protective devices. If these measures do not appear to be adequate, it may be advisable to design equipment with vacuum tubes rather than solid state components, if this is compatible with the intended use of the equipment.
11.35 A so-called “electromagnetic” shield consists of a continuous metal, e.g., steel, soft iron, or copper, sheet surrounding the system to be protected. Shielding of individual components or small subsystems is generally not practical because of the complexity of the task. Good shielding practice may include independent zone shields, several thin shields rather than one thick one, and continuous joints. The shield should not be used as a ground or return conductor, and sensitive equipment should be kept away from shield comers. Apertures in shields should be avoided as far as possible; doors should be covered with metal sheet so that when closed they form a continuous part of the whole shield, and ventilation openings, which cannot be closed, should be protected by special types of screens or waveguides. In order not to jeopardize the effectiveness of the shielding, precautions must be taken in connection with penetrations of the housing by conductors, such as pipes, conduits, and metal-sheathed cables (§ 11.59).
11.36 Recommendations for circuit layout include the use of common ground points, twisted cable pairs, system and intrasystem wiring in “tree” format (radial spikes), avoiding loop layouts and coupling to other circuits, use of conduit or cope trays, and shielded isolated transformers. The avoidance of ground return in cable shields is also recommended. Some procedures carry over from communications and power engineering whereas others do not.
11.37 From the viewpoint of EMP protection, cable design represents an extension of both shielding and circuit practices. Deeply buried (more than 3 feet underground) cables, shield layer continuity at splices, and good junction box contacts are desirable. Ordinary braid shielding should be avoided. Compromises are often made in this area in the interest of economy, but they may prove to be unsatisfactory.
11.38 Good grounding practices will aid in decreasing the susceptibility of a system to damage by the EMP. A “ground” is commonly thought of as a part of a circuit that has a relatively low impedance to the local earth surface. A particular ground arrangement that satisfies this definition may, however, not be optimum and may be worse than no ground for EMP protection. In general, a ground can be identified as the chassis of an electronic circuit, the “low” side of an antenna system, a common bus, or a metal rod driven into the earth. The last depends critically on local soil conditions (conductivity), and it may result in resistively coupled currents in the ground circuit. A good starting point for EMP protection is to provide a single point ground for a circuit cluster, usually at the lowest impedance element- the biggest piece of the system that is electrically immersed in the earth, e.g., the water supply system.
11.39 Various protective devices may be used to supplement the measures described above. These are related to the means commonly employed to protect radio and TV transmission antennas from lightning strokes and power lines from current surges. Examples are arresters, spark gaps, band-pass filters, amplitude limiters, circuit breakers, and fuses. Typically, the protective device would be found in the “EMP room” at the cable entrance to an underground installation, in aircraft antenna feeds, in telephone lines, and at power entry panels for shielded rooms. On a smaller scale, diodes, nonlinear resistors, silicon-controlled rectifier clamps, and other such items are built into circuit boards or cabinet entry panels.
11.40 Few of the devices mentioned above are by themselves sufficient as a complete solution to a specific problem because each has some limitation in speed of response, voltage rating, power dissipation capacity, or reset time. Hence, most satisfactory protective devices are hybrids. For example, a band-pass filter may be used preceding a lightning arrester. The filter tends to stretch out the rise time of the EMP, thus providing sufficient time for the arrester to become operative. In general, a hybrid protection device must be designed specially for each application.
TESTING
11.41 Because of the complexities of the EMP response, sole reliance can not be placed on predictions based on analysis. Testing is essential to verify analysis of devices, components, and complete systems early in the design stage. Testing also is the only known method that can be used to reveal unexpected effects. These may include coupling or interaction modes or weaknesses that were overlooked during the design. In some simple systems, non linear interaction effects can be analyzed numerically, but as a general rule testing is necessary to reveal them. As a result of the test, many of the original approximations can be refined for future analysis, and the data can improve the analytic capability for more complex problems. Testing also locates weak or susceptible points in components or systems early enough for economic improvement. After the improvements, testing confirms that the performance is brought up to standard. A complete system should be tested to verify that it has been hardened to the desired level; subsequent periodic testing will indicate if any degradation has resulted from environmental or human factors.
11.42 Since the cessation of atmospheric weapons tests, heavy reliance has been placed on simulation to test the EMP hardness of systems. The classes of EMP tests include: (1) low-level current mapping; (2) high-level current injection; (3) high-level electromagnetic fields. Low-level current mapping should be used at the beginning of any test program. With the system power turned off, the magnitudes and signatures on internal cables are determined in a low-level field. This provides an insight into the work that must follow. After indicated improvements are made, a high-level current can be injected directly into the system with the system power on to explore for nonlinearities, and to uncover initial indications of system effects. If subsystems malfunction, it may be desirable to conduct extensive subsystem tests in the laboratory. Finally, testing in a high-level electromagnetic field is essential.
11.43 The type of excitation must be defined in any type of test. The two principal choices are: (1) waveform simulations, which provide time domain data, and (2) continuous wave (CW) signals, which provide frequency-domain data. If the intent is to match a system analysis in the frequency domain to measured system response, CW signals may be the more suitable. If the test results were being compared to known electronic thresholds, it is frequently necessary to test in the time domain. Both types of tests should be considered for a complete analysis.
11.44 Large-scale simulators are required for the final test of large systems. The two principal kinds of large simulators are metallic structures that guide an electromagnetic wave past a test object, and antennas that radiate an electromagnetic field to the object. Each type of simulator may use either pulse generators (time domain) or CW signal generators (frequency domain). Pulse generators themselves can be either high-level single shot or low-level repetitive.
11.45 The essential elements of a guided-wave or transmission-line simulator include a pulser, a transition section, working volume, and a termination. An electromagnetic wave of suitable amplitude and wave shape is generated by the pulser. This wave is guided by a tapered section of transmission line (the transition section) from the small cross-sectional dimension of the pulser output to the working volume. The working volume, where the test object is located, should be large enough to provide a certain degree of field uniformity over the object. This condition is satisfied if the volume of the test object is about one-third (or less) that of the working volume, depending on the degree of field perturbation that is acceptable. The termination region prevents the reflection of the guided wave back into the test volume; it consists of a transition section that guides the incident wave to a geometrically small resistive load whose impedance is equal to the characteristic impedance of the transmission line structure.
11.46 The basic types of radiating simulators are long wire, biconical dipole, or conical monopole. The long wire is usually a long dipole oriented parallel to the earth's surface. It is supported above the ground by nonconducting poles with high-voltage insulators. The two arms of the dipole are symmetric about the center and constructed from sections of lightweight cylindrical conductor, such as irrigation pipe. Pipe sections decrease in diameter with increasing distance from the center, and resistors are placed between the pipe sections to shape the current wave and to reduce resonances. The two arms of the dipole are oppositely charged, and when the voltage across the spark gap at the dipole center reaches the breakdown voltage, the gap begins conducting and a wave front propagates away from the gap.
11.47 Conical and biconical antennas use pulsers, such as Marx generators or CW transmitters, instead of relying on the discharge of static surface charges. The antennas consist of light weight conducting surfaces or wire grids.
11.48 Electromagnetic scale modeling may sometimes be an important alternative to full-scale testing of a system. Because of the difficulty in introducing minute openings or poor bonds into models, and since these often control interior fields, the usefulness of modeling ordinarily is limited to the measurement of external fields, voltages, and currents. Once these parameters are known for a complex structure, perhaps having cable runs, analysis can often provide internal field quantities of interest.
EMP AND ELECTRIC POWER SYSTEMS
11.49 Some indication of the possible threat of the EMP to commercial electric power system may be obtained by considering the effects of lightning strokes and switching surges. In power systems, protection against lightning is achieved by means of overhead “ground” wires and lightning arresters of various types. By providing an effective shunt, an overhead ground can divert most of the lightning surge from the phase conductors. Such grounding, however, would afford only partial protection from the EMP. Furthermore, although there are some similarities between the consequences of lightning and those of the EMP, there are differences in the nature of the current (or voltage) pulse which make the lightning arresters in common use largely ineffective for the EMP.
11.50 The general manner of the growth and decay of the current induced by the EMP from a high-altitude burst in an overhead transmission line is indicated by the calculated curve in Fig. 11.50 The details of the curve will vary with the conditions, but the typical features of the current pulse are as shown: a very rapid rise to a peak current of several thousand amperes in a fraction of a microsecond followed by a decay lasting up to a millisecond for a long transmission line. The current surge in an overhead power line caused by a lightning stroke increases to a maximum more slowly and persists for a longer time than for the EMP. As a result, older conventional lightning arresters are less effective for the EMP from high-altitude explosions than for lightning. Modern lightning arresters, however, can provide protection against EMP in many applications and hybrid arresters (§ 11.40) are expected to be even better.
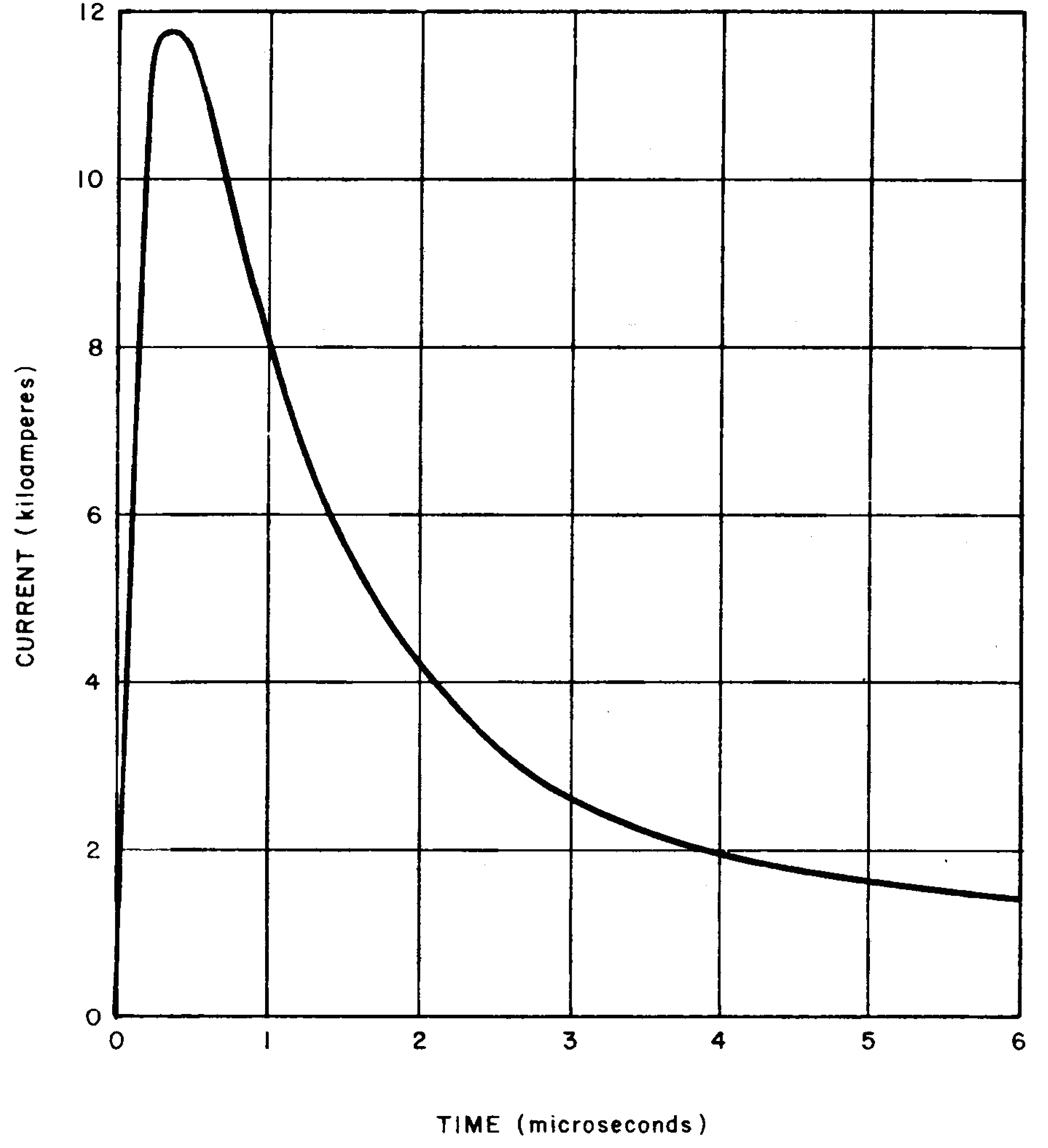
11.51 In the absence of adequate protection, the surge voltages on overhead power lines produced by the EMP could cause insulator flashover, particularly on circuits of medium and low voltage. (The components of high-voltage transmission systems should be able to withstand the EMP surge voltages.) If flashovers occur in the event of a high altitude burst many would be experienced over a large area. Such simultaneous multiple flashovers could lead to system instability.
11.52 Switching surges occur when power lines are energized or de-energized. In systems of moderate and low voltage such surges can cause breakers in the switching circuit to operate erroneously, but the effect of the EMP is uncertain because the current rise in a switching surge is even slower than for lightning. In extra-high-voltage (EHV) lines, i.e., 500 kilovolts or more, switching surges are accompanied by a rapidly increasing radiated electromagnetic field similar to that of the EMP. The currents induced in control and communications cables are sufficient to cause damage or malfunction in associated equipment. The information obtained in connection with the development of protective measures required for EHV switching stations should be applicable to EMP protection.
11.53 There is a growing movement in the electric power industry to substitute semiconductor devices for vacuum tubes in control and communications circuits. Solid-state components are, however, particularly sensitive to the EMP. Even a small amount of energy received from the pulse could result in erroneous operation or temporary failure. Computers used for automatic load control would be particularly sensitive and a small amount of EMP energy, insufficient to cause permanent damage, could result in faulty operation or temporary failure. Special attention is thus required in the protection of such equipment.
EMP AND RADIO STATIONS
11.54 Unless brought in underground and properly protected, power and telephone lines could introduce substantial amounts of energy into radio (and TV) stations. A major collector of this energy, however, would be the transmitting (or receiving) antennas since they are specially designed for the transmission and reception of electromagnetic energy in the radiofrequency region. The energy collected from the EMP would be mainly at the frequencies in the vicinity of the antenna design frequency.
11.55 Antenna masts (or towers) are frequently struck by lightning and spark gaps are installed at the base of the tower to protect the station equipment. But the gaps in common use, like those in power lines, are not very effective against the EMP. Actually, when an antenna is struck by lightning, the supporting guy wires, rather than the spark gaps, serve to carry most of the lightning current to the ground. Although the guy wires have insulators along their length, arcing occurs across them thereby providing continuity for the current. This flashover of the insulators would not, however, provide protection against the EMP. In fact, the guy wires would then serve as additional collectors of the EMP energy by induction.
11.56 In spite of protective devices, both direct and indirect, damage to radio stations by lightning is not rare. The most commonly damaged component is the capacitor in the matching network at the base of the antenna; it generally suffers dielectric failure. Capacitors and inductors in the phasor circuit are also subject to damage. It is expected that high-voltage capacitors would be sensitive to damage by the EMP. Such damage could result in shortening of the antenna feed line to the ground across the capacitor, thus precluding transmission until the capacitor is replaced. Experience with lightning suggests that there may also be damage to coaxial transmission lines from dielectric flashover. Solid-state components, which are now in common use in radio stations, would, of course, be susceptible to damage by the EMP and would need to be protected.
11.57 Radio transmitting stations employ various means to prevent interference from their own signals. These include shielding of audio wiring and components with low-level signals, single-point grounding, and the avoidance of loops. Such practices would be useful in decreasing the EMP threat.
EMP AND TELEPHONE SYSTEMS
11.58 Some of the equipment in telephone systems may be susceptible to damage from the EMP energy collected by power supply lines and by the subscriber and trunk lines that carry the signals. Various lightning arresting devices are commonly used for overhead telephone lines, but they may provide limited protection against the EMP un less suitably modified. Steps are being taken to improve the ability of the long-distance telephone network in the United States to withstand the EMP as well as the other effects of a nuclear explosion.
11.59 In a properly “hardened” system, coaxial cables are buried underground and so also are the main and auxiliary repeater or switching centers. In the main (repeater and switching) stations, the building is completely enclosed in a metal EMP shield. Metal flashing surrounds each metallic line, e.g., pipe, conduit, or sheathed cable, entering or leaving the building, and the flashing is bonded to the line and to the shield. Where this is not possible, protectors or filters are used to minimize the damage potential of the EMP surge. Inside the building, connecting cables are kept short and are generally in straight runs. An emergency source of power is available to permit operation to continue in the event of a failure (or disconnection) of the commercial power supply. The auxiliary (repeater) stations, which are also underground, do not have exterior shielding but the electronic equipment is protected by steel cases.
THEORY OF THE EMP2
DEVELOPMENT OF THE RADIAL ELECTRIC FIELD
11.60 The energies of the prompt gamma rays accompanying a nuclear explosions are such that, in air, Compton scattering is the dominant photon interaction (see Fig. 8.97b). The scattered photon frequently retains sufficient energy to permit it to repeat the Compton process. Although scattering is somewhat random, the free electrons produced (and the scattered photons) tend, on the average, to travel in the radial direction away from the burst point. The net movement of the electrons constitutes an electron current, referred to as the Compton current. The prompt gamma-ray pulse increases rapidly to a peak value in about 10-8 seconds or so, and the Compton current varies with time in a similar manner.
11.61 When the electrons are driven radially outward by the flux of gamma rays, the atoms and molecules from which they have been removed, i.e., the positive ions, travel outward more slowly. This results in a partial separation of charges and a radial electric field. The lower energy (secondary) electrons generated by collisions of the Compton electrons are then driven back by the field toward the positive charges. Consequently, a reverse electron current is produced and it increases as the field strength increases. This is called the “conduction current” because, for a given field strength, its magnitude is determined by the electrical conductivity of the ionized medium. The conductivity depends on the extent of ionization which itself results from the Compton effect; hence the conductivity of the medium will increase as the Compton current increases. Thus, as the radial field grows in strength so also does the conduction current. The conduction current flows in such a direction as to oppose this electric field; hence at a certain time, the field will cease to increase. The electric field is then said to be “saturated.” At points near the burst, the radial electric field reaches saturation sooner and is somewhat stronger than at points farther away.
11.62 In a perfectly homogeneous medium, with uniform emission of gamma rays in all directions, the radial electric field would be spherically symmetrical. The electric field will be confined to the region of charge separation and no energy will be radiated away. In a short time, recombination of charges in the ionized medium occurs and the electric field strength in all radial directions decreases within a few microseconds. The energy of the gamma rays deposited in the ionized sphere is then degraded into thermal radiation (heat). If the symmetry of the ionized sphere is disturbed, however, nonradial oscillations will be initiated and energy will be emitted as a pulse of electromagnetic radiation much of which is in the radio frequency region of the spectrum. Since, in practice, there is inevitably some disturbance of the spherical symmetry in a nuclear explosion, all such explosions are accompanied by a radiated EMP, the strength of which depends on the circumstances.
GENERAL CHARACTERISTICS OF THE EMP
11.63 The radiation in the EMP covers a wide range of frequencies with the maximum determined by the rise time of the Compton current. This is typically of the order of 10-8 second and the maximum frequency for the mechanism described above is then roughly 108 cycles per second, i.e., 108 hertz or 100 megahertz. Most of the radiation will, however, be emitted at lower frequencies in the radiofrequency range. The rise time is generally somewhat shorter for high-altitude bursts than for surface and medium-altitude bursts; hence, the EMP spectrum in high-altitude bursts tends toward higher frequencies than in bursts of the other types.
11.64 The prompt gamma rays from a nuclear explosion carry, on the average, about 0.3 percent of the explosion energy (Table 10.138) and only a fraction of this, on the order of approximately 10-2 for a high-altitude burst and 10-7 for a surface burst, is radiated in the EMP. For a 1-megaton explosion at high altitude, the total energy release is 4.2 × 1022 ergs and the amount that is radiated as the EMP is roughly 1018 ergs or 1011 joules. Although this energy is distributed over a very large area, it is possible for a collector to pick up something on the order of 1 joule (or so) of EMP energy. The fact that a small fraction of a joule, received as an extremely short pulse, could produce either permanent or temporary degradation of electronic devices, shows that the EMP threat is a serious one.
11.65 Although all nuclear bursts are probably associated with the EMP to some degree, it is convenient to con sider three more-or-less distinct (or extreme) types of explosions from the EMP standpoint. These are air bursts at medium altitudes, surface bursts, and bursts at high altitudes. Medium-altitude bursts are those below about 19 miles in which the deposition region does not touch the earth's surface. The radius of the sphere ranges roughly from 3 to 9 miles, increasing with the burst altitude. The EMP characteristics of air bursts at lower altitudes, in which the deposition region does touch the earth, are intermediate between median-altitude and surface bursts. At burst altitudes below about 1.2 miles, the radiated pulse has the general characteristics of that from a surface burst.
MEDIUM-ALTITUDE AIR BURSTS
11.66 In an air burst at medium altitude, the density of the air is somewhat greater in the downward than in the upward direction. The difference in density is not large, although it increases with the radius of the deposition (or source) region, i.e., with increasing altitude. The frequency of Compton collisions and the ionization of the air will vary in the same manner as the air density. As a result of the asymmetry, an electron current is produced with a net component in the upward direction, since the symmetry is not affected in the azimuthal (radial horizontal) direction. The electron current pulse initiates oscillations in the ionized air and energy is emitted as a short pulse of electromagnetic radiation. The EMP covers a wide range of frequencies and wave amplitudes, but much of the energy is in the low-frequency radio range. In addition, a high-frequency pulse of short duration is radiated as a result of the turning of the Compton electrons by the earth's magnetic field (§ 11.71).
11.67 The magnitude of the EMP field radiated from an air burst will depend upon the weapon yield, the height of burst (which influences the asymmetry due to the atmospheric density gradient), and asymmetries introduced by the weapon (including auxiliary equipment, the case, or the carrying vehicle). At points outside the deposition region, for the lower-frequency EMP arising from differences in air density, the radiated electric field E( t) at any specified time t as observed at a distance R from the burst point is given by
where is the radius of the deposition region, $E_0(t)$ is the radiated field strength at the distance $R_0$, i.e., at the beginning of the radiating region, at the time $t$, and θ is the angle between the vertical and a line joining the observation point to the burst point. It follows from equation (11.67.1) that, as stated in § 11.06, the EMP field strength is greatest in directions perpendicular to the (vertical) electron current. Values of $E_0(t)$ and are determined by computer calculations for specific situations; $E_0(t)$ is commonly from a few tens to a few hundred volts per meter and $R_0$ is from about 3 to 9 miles (§ 11.09). The interaction of the gamma rays with air falls off roughly exponentially with distance; hence, the deposition region does not have a precise boundary, but $R_0$ is taken as the distance that encloses a volume in which the conductivity is 10-7 mho per meter or greater.
SURFACE BURSTS
11.68 In a contact surface burst, the presence of the ground introduces a strong additional asymmetry. Compared with air, the ground is a very good absorber of neutrons and gamma rays and a good conductor of electricity. Therefore, the deposition region consists approximately of a hemisphere in the air and there is a net electron current with a strong component in the upward direction. Further, the conducting ground provides an effective return path for the electrons with the result that current loops are formed. That is, electrons travel outward from the burst in the air, then return toward the burst point through the higher conductivity ground. These current loops generate very large azimuthal magnetic fields that run clockwise around the burst point (looking down on the ground) in the deposition region, especially close to the ground (Fig. 11.10). At points very near the burst, the air is highly ionized and its conductivity exceeds the ground conductivity. The tendency for the conduction current to shift to the ground is therefore reduced, and the magnetic fields in the ground and in the air are decreased correspondingly.
11.69 Large electric and magnetic fields are developed in the ground which contribute to the EMP, in addition to the fields arising from the deposition region. As a result of the number of variables that can affect the magnitude and shape of the fields, it is not possible to describe them in a simple manner. The peak radiated fields at the boundary of the deposition region are ten to a hundred times stronger in a direction along the earth than for a similar air burst. The variation with distance of the peak radiated electric field along the earth's surface is given by
where $E_0$ is the peak radiated field at the radius $R_0$ of the deposition region and E is the peak field at the surface distance $R$ from the burst point. For observation points above the surface the peak radiated field falls off rapidly with increasing distance. As stated in § 11.12, $R_0$ is roughly 2 to 5 miles, depending on the explosion yield; E0 may be several kilovolts per meter.
HIGH-ALTITUDE BURSTS
11.70 The thickness and extent of gamma-ray intensity decreases with half of the deposition region for bursts of 1 and 10 megatons yield, respectively, for various heights of burst (HOB) are shown in Figs. 11.70a and b. The abscissas are distances in the atmosphere measured parallel to the earth's surface from ground zero. The curves were computed from the estimated gamma-ray emissions from the explosions and the known absorption coefficients of the air at various densities (or altitudes). At small ground distances, i.e., immediately below the burst, the deposition region is thicker than at larger distances because the gamma-ray intensity decreases with distance from the burst point. Since the gamma rays pass through air of increasing density as they travel toward the ground, most are absorbed in a layer between altitudes of roughly 40 and 10 miles.
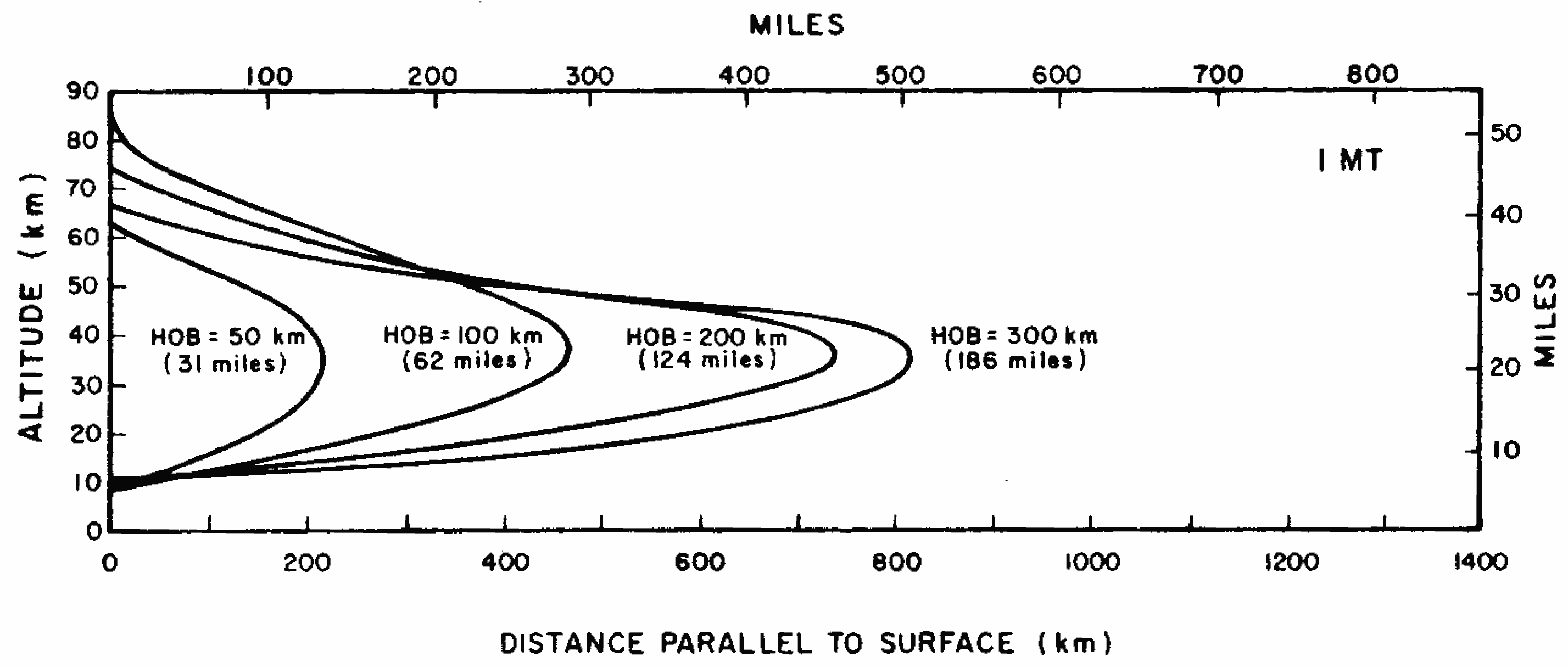
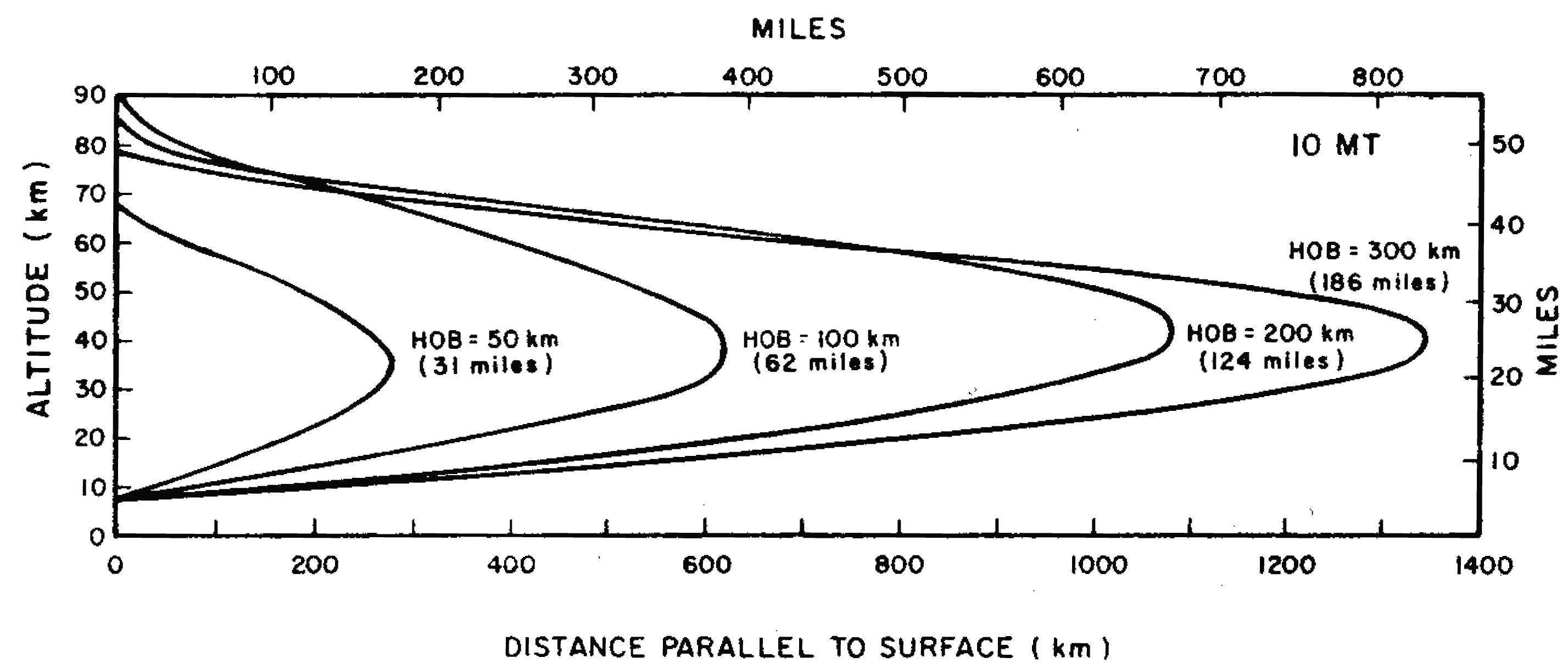
11.71 Unless they happen to be ejected along the lines of the geomagnetic field, the Compton electrons resulting from the interaction of the gamma-ray photons with the air molecules and atoms in the deposition region will be forced to follow curved paths along the field lines.3 In doing so they are subjected to a radial acceleration and the ensemble of turning electrons, whose density varies with time, emits electromagnetic radiations which add coherently. The EMP produced in this manner from a high-altitude burst—and also to some extent from an air burst—is in a higher frequency range than the EMP arising from local asymmetries in moderate-altitude and surface bursts (§ 11.63).
11.72 The curves in Figs. 11.70a and b indicate the dimensions of the deposition (source) region, but they do not show the extent of coverage on (or near) the earth's surface. The EMP does not radiate solely in a direction down from the source region; it also radiates from the edges and at angles other than vertical beneath this region. Thus, the effect at the earth's surface of the higher-frequency EMP extends to the horizon (or tangent point on the surface as viewed from the burst). The lower frequencies, however, will extend even beyond the horizon because these electromagnetic waves can follow the earth's curvature (cf. § 10.92). Table 11.72 gives the distances along the surface from ground zero to the tangent point for several burst heights.
Burst Altitude (miles) | Tangent Distance (miles) |
---|---|
62 | 695 |
93 | 850 |
124 | 980 |
186 | 1,195 |
249 | 1,370 |
311 | 1,520 |
11.73 The peak electric field (and its amplitude) at the earth's surface from a high-altitude burst will depend upon the explosion yield, the height of burst, the location of the observer, and the orientation with respect to the geomagnetic field. As a general rule, however, the field strength may be expected to be tens of kilovolts per meter over most of the area receiving the EMP radiation. Figure 11.73 shows computed contours for Emax, the maximum peak electric field, and various fractions of Emax for burst altitudes between roughly 60 and 320 miles, assuming a yield of a few hundred kilotons or more. The distances, measured along the earth's surface, are shown in terms of the height of burst. The spatial distribution of the EMP electric field depends on the geomagnetic field and so varies with the latitude; the results in the figures apply generally for ground zero between about 30° and 60° north latitude. South of the geomagnetic equator the directions indicating magnetic north and east in the figure would become south and west, respectively. It is evident from Fig. 11.73 that over most of the area affected by the EMP the electric field strength on the ground would exceed 0.5 Emax For yields of less than a few hundred kilotons, this would not necessarily be true because the field strength at the earth's tangent could be substantially less than 0.5 Emax.
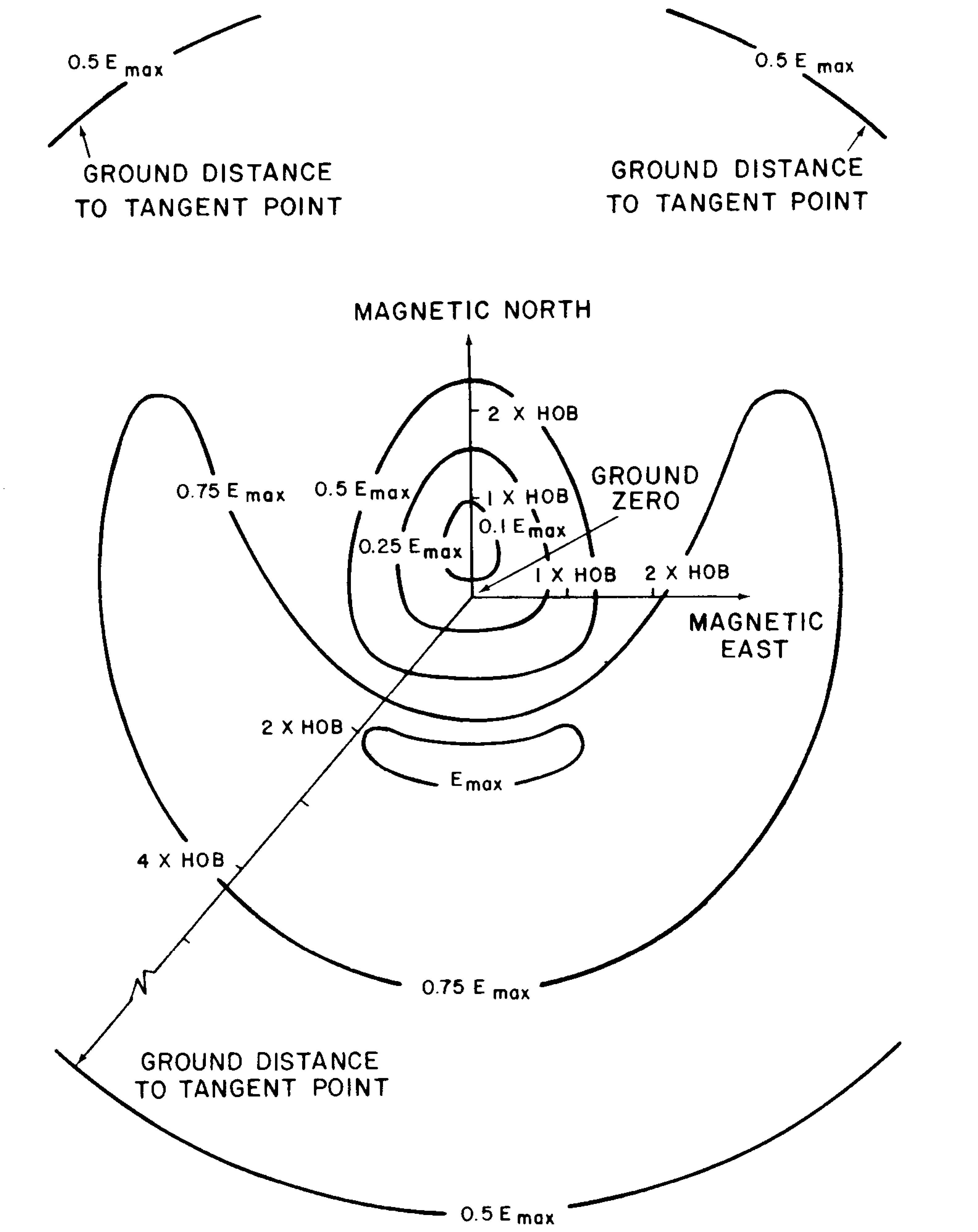
11.74 The reason why Fig. 11.73 does not apply at altitudes above about 320 miles is that at such altitudes the tangent range rapidly becomes less than four times the height of burst. The distance scale in the figure, in terms of the HOB, then ceases to have any meaning. For heights of burst above 320 miles, a set of contours similar to those in Fig. 11.73 can be plotted in terms of fractions of the tangent distance.
11.75 The spatial variations of EMP field strength arise primarily from the orientation and dip angle of the geomagnetic field, and geometric factors related to the distance from the explosion to the observation point. The area of low field strength slightly to the north of ground zero in Fig. 11.73 is caused by the dip in the geomagnetic field lines with reference to the horizontal direction. Theoretically, there should be a point of zero field strength in the center of this region where the Compton electrons would move directly along the field lines without turning about them, but other mechanisms, such as oscillations within the deposition region, will produce a weak EMP at the earth's surface. The other variations in the field strength at larger ground ranges are due to differences in the slant range from the explosion.
11.76 The contours in Fig. 11.73 apply to geomagnetic dip angles of roughly 50° to 70°. Although Emax would probably not vary greatly with the burst latitude, the spatial distribution of the peak field strength would change with the dip angle. At larger dip angles, i.e., at higher latitudes than about 60°, the contours for Emax and 0. 75 Emax would tend more and more to encircle ground zero. Over the magnetic pole (dip angle 90°), the contours would be expected theoretically to consist of a series of circles surrounding ground zero, with the field having a value of zero at ground zero. At lower dip angles, i.e., at latitudes less than about 30°, the tendency for the contours to become less circular and to spread out, as in Fig. 11.73, would be expected to increase.
BIBLIOGRAPHY
- et al., “EMP Seal Evaluation,” Physics International Co., San Leandro, California, January 1971.
- , “EMP Directory for Shelter Design,” Illinois Institute of Technology Research Institute, Chicago, Illinois, April 1968.
- , “EMP Threat and Countermeasures for Civil Defense Systems,” Illinois Institute of Technology Research Institute, Chicago, Illinois, November 1968.
- *"Electromagnetic Pulse Problems in Civilian Power and Communications," Summary of a seminar held at Oak Ridge National Laboratory, August 1969, sponsored by the U.S. Atomic Energy Commission and the Department of Defense/Office of Civil Defense.
- "Electromagnetic Pulse Sensor and Simulation Notes, Volumes 1-10," Air Force Weapons Laboratory, April 1967 through 1972, AFWL EMP 1–1 through 1–10.
- "EMP Protection for Emergency Operating Centers," Department of Defense/Office of Civil Defense, May 1971, TR–61–A.
- "EMP Protective Systems," Department of Defense/Office of Civil Defense, November 1971, TR–61–B.
- "EMP Protection for AM Radio Broadcast Stations," Department of Defense/Office of Civil Defense, May 1972, TR–61–C.
- , “Operation Survival,” Bell Laboratories Record, January 1969, page 11.
- , “The Development of a Radio Signal from a Nuclear Explosion in the Atmosphere,” J. Geophys. Res., 73, 405 (1968).
- , “Absence of Electromagnetic Pulse Effects on Monkeys and Dogs,” J. Occupational Medicine, 14, 380 (1972).
- , “Detection of Electromagnetic Radiation from Nuclear Explosions in Space,” Phys. Rev. 137, B1369 (1965).
- , “Experimental Results of Testing Resistors Under Pulse Conditions,” Electrical Standards Division 2412, Sandia Laboratory, Albuquerque, New Mexico, November 1967.
- , Program Coordinator, “DNA EMP Awareness Course Notes,” 2nd ed., Illinois Institute of Technology Research Institute, Chicago, Illinois, August 1973, DNA 2772T.
- , “Effects of Nuclear EMP on AM Broadcast Stations in the Emergency Broadcast System,” Oak Ridge National Laboratory, July 1971, ORNL–TM–2830.
- , “EMP Impact on U.S. Defenses,” Survive, 2, No. 6, 2 (1969).
- , “A Program to Counter Effects of Nuclear EMP in Commercial Power Systems,” Oak Ridge National Laboratory, October 1972, ORNL–TM–3552.
- Ricketts, L. W., et al. , “EMP Radiation and Protective Techniques,” Wiley–Interscience, 1976.
- et al., “Late Time Source for Close-In EMP,” Science Applications, La Jolla, California, August 1972, DNA 3064F, SAl–72–556––L–J.
ENDNOTES
- 1 This section (§§ 11.27 through 11.59) is of particular interest to electrical and electronic engineers. [ref. Section 2] X
- 2 The remainder of this chapter may be omitted without loss of continuity. [ref. Section 3] X
- 3 At higher altitudes, when the atmospheric density is much less and collisions with air atoms and molecules are less frequent, continued turning of the electrons (beta particles) about the field lines leads to the helical motion referred to in §§ 2.143, 10.27. [ref. § 11.71] X